The pressure to bring new products to the market, with reduced time to market, high quality and reduced cost, has never been greater. For most electrical and electronic products, the necessity of complying with electromagnetic compatibility (EMC) regulations in order to sell in global markets adds to these pressures. Considering the increased importance of EMC testing for electronic systems, the associated challenges seem to grow simultaneously. These challenges range from increased importance of EMC in the product design phase, to improving EMC test standards for new technologies to developing more efficient instrumentation for EMC testing.
The RF immunity standard IEC-61000-4-3 specifies a requirement to generate a uniform field at a test distance of 3m between the tip of antenna and the device under test (DUT). Such an area with a uniform field is termed a quiet zone in the EMC industry. A certain level of E-field is applied to the DUT, placed in the quiet zone for immunity testing. The area of uniform illumination is 1.5 by 1.5 meters to ensure that portions of any interconnecting cable to the DUT is illuminated by the field. The field is deemed uniform when 75 percent of 12 points in this area comply with the 0 to +6 dB rule [1]. In RF immunity systems using traditional design techniques, the designer has to analyze a large number of individual specifications of the system components and take into considerations for their integration into the test system.
Conventional Setup
A typical radiated immunity (RI) test setup consists of many components including antennas, amplifier, signal generator, power meters, directional couplers and E-field probe. Figure 1 presents a typical RI setup according to IEC 61000-4-3. The DUT is placed on the turntable inside the chamber and is placed 3m away from the tip of the antenna [1]. Typically, long runs of RF cables are used to connect from the amplifier output to the input of the dual directional coupler (DDC), from the coupler’s output to the feed-through connector on the chamber wall, and from the chamber feed-through to the antenna input.
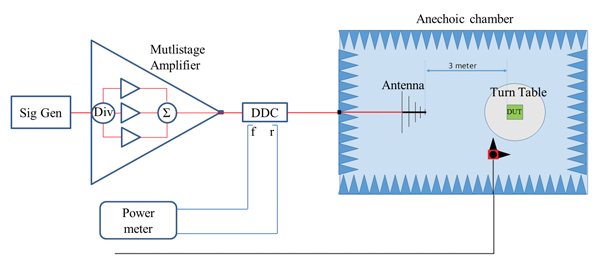
The power amplifier is one of the most expensive components in an EMC test system. However, in the conventional EMC RI setup such as the one shown in Figure 1, up to 3-6 dB of an amplifier’s rated power is lost at multiple stages in the setup itself (see Table 1).
Typical loss values (dB) |
|||||
Freq (GHz) |
Combiner - From Our Sponsors -
|
Coupler |
RF cable |
Total Loss |
|
3m cable |
10m cable |
||||
1 |
0.6-0.8 |
0.2-0.4 |
0.1 dB/m |
1.1-1.5 |
1.8-2.2 |
6 |
1.5-1.8 |
0.5-0.8 |
0.25 dB/m |
2.75-3.35 |
4.85-5.1 |
Table 1: Loss values in an RI test setup
The power loss in the test setup mainly comes from the connecting cables between different parts in the setup. Such pieces of cable from the amplifier output to the input of the DDC, between the coupler’s output and feed-through on the chamber wall, and from the chamber feed-through to the antenna input. The amplifier itself also adds to these losses as it consists of multiple stages and a combiner which eventually combines the output of the multiple stages. In addition, the frequency dependent losses of the RF cables exacerbate the situation.
Therefore, on one hand EMC standards specify the test setup requirements in terms of E-field strengths within the quiet zone, while on the other hand such power losses force us to design systems in terms of amplifier rated power and safety margins. Eventually, a much higher rated power amplifier is required to overcome the power loss occurring from the inefficient test setup.
A typical alternate approach used to reduce such losses is to put the immunity rack inside the test chamber. This approach is not only non-compliant with the standard, but it can also impact the immunity measurement results.
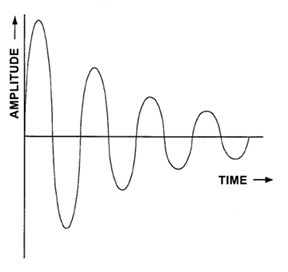
Field Generators
The Concept
To achieve high output power, the traditional approach is to combine power from small amplifiers, and then this high power is supplied to a single antenna through a path comprising of a directional coupler and a long RF cable.
The proposed model is comprised of active antenna arrays, integrated amplifiers, and directional couplers. Each antenna element in the array has its own amplifier and directional coupler. Instead of adding power in a multi-stage amplifier, field generators combine the E-fields generated by each chain of antenna and amplifier. The amplifiers and antennas are coupled together in the design. Therefore, such field generators not only remove the internal loss within multiple stages of the amplifier rack, but also remove the losses due to the cabling within the amplifier and antenna themselves.
In the following sections, the design of such field generators for the RI test setup in compliance with IEC 61000-4-3 is presented with simulation and measurement results.
Design Considerations
There are several considerations which need to be kept in mind while designing such field generators, the important items are discussed in this section.
Antenna selection
Log-periodic dipole array (LPDA) antennas used in broadband applications can achieve high directivity and low cross-polarization ratio over a very large frequency range. Such wideband antennas have typically been constructed using metallic booms and dipole radiating elements. In applications where space and weight is restricted, antennas need to be light-weight and have a small physical size and increase frequency.
Microstrip antennas that operate as a single element usually have a relatively large half power beamwidth, low gain and low radiation efficiency. In order to improve on these parameters, microstrip antennas are used in array configurations to increase the gain and range of the radiating structure [2]. There are many effects such as mutual coupling between elements which must be taken into consideration when analyzing an array structure. As a result, full wave analyses are usually used to model arrays.
The log periodic antenna structure is similar to a proximity coupled antenna; however, the elements are designed such that they follow logarithmic sizing and spacing [3]. These structures have relatively broad bandwidth. The antenna array in such field generator is comprised of three log-periodic antennas. The antenna array has been designed and simulated in software specifically designed for the purpose. The simulation model of the designed array is shown in Figure 3. The LPDA antennas have 29 elements, resulting in a very low “ripple” on the frequency response. The spacing between the antenna array elements has been optimized for gain and phase matching.
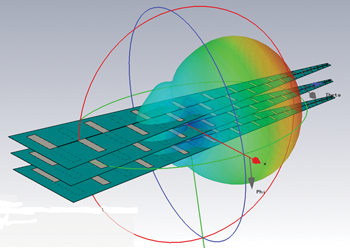
The far field realized gain polar plots of the simulated antenna array are shown in Figure 4, with some results summarized in Table 2. It has been shown that the gain of the antenna array is slowly increasing from 1 GHz to 6 GHz (around 11 dB @ 1 GHz and 13 dB @ 6 GHz).
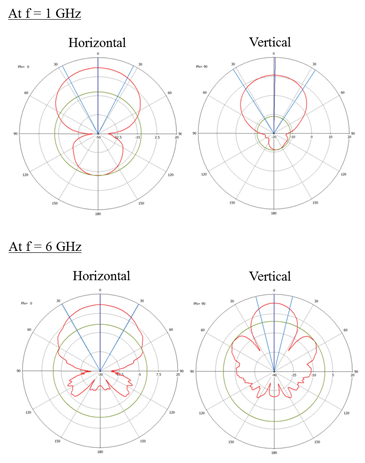
At f = 1GHz |
At f = 6 GHz |
|||
Horizontal Pol. |
Vertical |
Horizontal Pol. |
Vertical |
|
Main lobe mag. |
11 dB |
11 dB |
13.1 dB |
13.1 dB |
Angular width (3dB) |
53.8° |
65.7° |
59° |
27° |
Side lobe level |
-22.1 dB |
-21.8 dB |
-12.8 dB |
-14.1 dB |
~30°x30° |
~20°x30° |
Table 2: Simulation results of LPDA antenna array
Amplifier Selection
For a trouble-free and long life operation of power semiconductor devices (such as BJT, MOSFET, IGBT), it is critical to keep the overall power dissipation of the device within a safe operating area (SOAR) [4]. In this regard, much emphasis is given on choosing the optimal class of the amplifier for heat generation and dissipation. The class of the amplifier mainly influences its overall efficiency and the load driving capability of an amplifier’s output stage. Theoretically, in Class A amplifiers 50 percent of the power is heat whereas in Class AB designs it is only 12.5 percent. Class A amplifiers can achieve efficiency from only 20 percent to 30 percent in reality as compared to 60 percent efficiency in the case of class AB. The Class AB amplifier is chosen for such field generators as it offers the higher efficiency for given output levels.
A field generator presented here has three amplifiers, one for each antenna. Each amplifier has three amplifiers stages, where the final stage is a GaN amplifier. Each amplifier module has its own microcontroller, in order to control all amplifier parameters independently for each amplifier module (bias currents, protections, amplifier gain etc.).
Apart from this, each amplifier module has its own coupler, forward power meter and reflected power meter. The gain of each amplifier can be adjusted independently to ensure all antennas are fed with the same RF power. Also, the phase difference between all amplifiers has to be minimized to maintain the desired beam shape.
Figure 5 shows a graph for the overall frequency response from the input connector (at the power supply side) to the output of one of the amplifiers, including a 3 meter N type cable. As can be seen, the frequency response has a slight up tilt at the higher frequency end. This compensates for the frequency response of longer N-type cables.
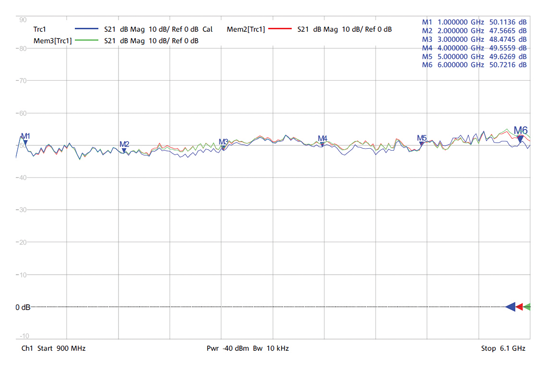
Integration of the parts
The rectangular metallic housing at the back side is designed as part of the overall radiating element. Therefore, this block does not negatively affect the antenna array performance. A single coaxial cable performs a dual function. It carries RF input signal to the amplifier, and it also carries the DC power supply signal to power up the electronic circuitry inside the unit. Figure 6 shows the complete assembly of a field generator design.
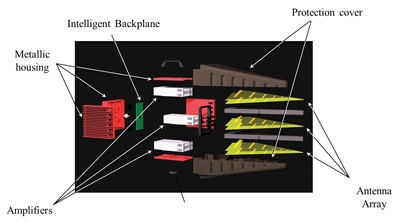
Proposed RI Test Setup
The new proposed setup with a field generator comes with a slightly different setup than a traditional setup. It is still in full compliance with IEC 61000-4-3. The new setup uses less equipment, and is thus less complex and requires less installation time.
Figure 7 shows the new setup for RI testing according to IEC 61000-4-3. The field generator is placed inside the chamber at a 3 meter distance from the DUT. Our company’s proprietary modular platform is used as the main supporting equipment in the new setup. This platform has seven slots for plugin cards, thus is capable of providing seven functionalities at the same time. In the current setup, the platform is configured to be used as a signal generator, power meter, and power supply for the field generator.
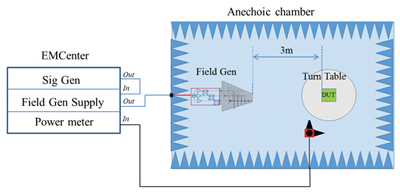
The field uniformity tests have been performed at 10 V/m to validate the performance and to ensure sufficient linear behavior of the system. In order to determine if the system produces enough field level, the available power of the system must be at least 5.1 dB (factor 1.8) higher than the highest recorded power of the 16 points at each frequency. This is because, although the validation is performed using CW signals, the standard requires the final tests be performed with a 80 percent amplitude modulation applied. According to IEC 61000-4-3, the available power may be determined at 2 dB compression of the output. The available power of the field generator is 10W per amplifier or 30W in total (44.7 dBm) which means the maximum power in the measurement should not exceed 44.7 dBm – 5.1 dB = 39.6 dBm so that the system has enough headroom to allow for the 80 percent amplitude modulation.
The red line in both the graphs in Figure 8 represents the maximum error limit for 6dB error. The error graph for 75 percent of the measured points in the vertical and horizontal planes are shown in Figure 8. It shows that 12 out of 16 points are within the specified 6 dB error limit.
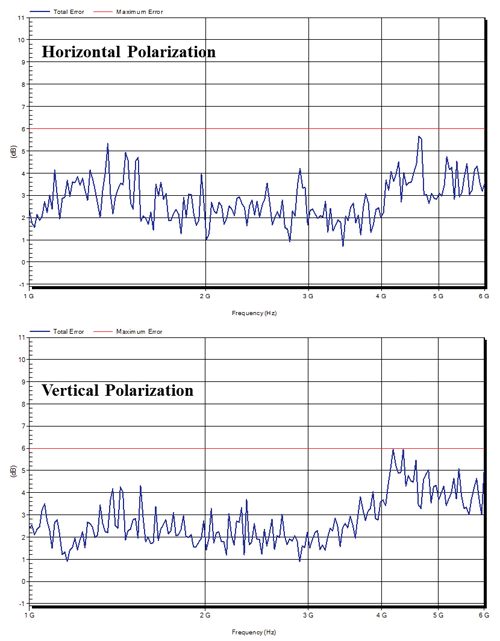
Conclusion
In this article, the concept of field generators is presented and validated as per the RI testing requirements specified in IEC 61000-4-3, i.e. 10V/m at a 3m distance between the DUT and the tip of the antenna. They simplify the test setup in terms of setup complexity and also allow easy installation. The concept is scalable to much higher field strengths and different frequency ranges with design modifications, e.g. varying the number of antenna array elements and modifying the integrated amplifier design. Such field generators not only introduce a new instrument category in the world of EMC testing but also provide a cost-effective solution to the existing challenges in RI testing.
Acknowledgements
This article is based on a paper originally presented at EMC Turkiye 2015, held September 2-4, 2015 at Isik University, Masklak, Istanbul.
The authors wish to express their gratitude to Patrick Dijkstra, Zhong Chen and Janet O’ Neil for their exemplary guidance, valuable feedback and constant encouragement throughout the process of writing this article.
References
- IEC 61000-4-3 Electromagnetic compatibility (EMC)-Part 4-3: “Testing and measurement techniques-Radiated, radio-frequency, electromagnetic field immunity test”, 3rd Edition, February 2006.
- C. A. Balanis, “Antenna Theory: Analysis and Design”, 3rd Edition, Hoboken, NJ: Wiley, 2005.
- D. M. Pozar and D. H. Schubert, “Microstrip Antennas—The Analysis and Design of
Microstrip Antennas and Arrays”, New York: IEEE Press, 1995. - Thomas L. Floyd, “Digital Electronic Fundamentals”, 8th Edition, Prentice Hall,
July 2009, NJ. - James Colotti, “EMC Design Fundamentals,” Systems, Applications and Technology Conference, 2006. LISAT 2006. IEEE Long Island.
Ammar Sarwar is a support engineer for ETS-Lindgren, based in the company’s Taufkirchen, Germany office, where he works on test and measurement projects for automotive, wireless, consumer electronics, and defense applications. He received his Bachelor of Science degree in electrical engineering from the National University of Science and Technology, Islamabad, Pakistan and his Master of Science degree in communications engineering, with an emphasis on RF and EMC, from the Technical University Munich, Germany. Before joining ETS-Lindgren, he worked in the EMC department of BMW AG Munich (on behalf of CSA Group Bayern) where we was involved in EMC design/simulation and EMC testing of automotive components in RF shielded chambers. His areas of interest include EM simulation tools for improved RF design and test measurements. Sarwar can be reached at ammar.sarwar@ ets-lindgren.de.
Vincent Keyser is the Regional Sales Director, EMEA South, for ETS-Lindgren in Europe. He has over 10 years of experience in developing RF test solutions for EMC and associated technical sales. He holds an MSEE degree in RF Engineering from the Institut National des Sciences Appliquées (INSA), Rennes, France. Since joining ETS-Lindgren in 2011, his focus has been on supporting customers through direct interaction with the company’s engineering, manufacturing and sales personnel. His areas of interest include complete test and measurement systems for EMC and MIMO/OTA automotive and wireless device applications, respectively. Keyser can be reached at vincent.keyser@ets-lindgren.de.